OK, Dr. Z – you’ve been talking about a lot of different isotopes and only a few of them are natural. Where do all the rest of them come from?
Surprisingly, they’re grown on a farm in the Midwest! Well…OK…maybe not. But wouldn’t it be nice if it were that easy. Actually, from your standpoint it is about that easy – you can go to a catalog, pick out what you need (or what strikes your fancy), and place an order. The company will confirm that you actually have a radioactive materials license that lets you have what you’d like to order, you pay them, they ship your sources, and Bob’s your uncle! If your license does not let you posses the nuclide(s) you’re looking for in the activity you requested then you’ll have to get your license amended before the vendor is allowed to sell and ship the material to you. I should also mention that it can sometimes take a while to actually take delivery of the sources – one project I was working on ordered a custom source that took over 6 months to fabricate and test, many sources are only produced overseas, and some are produced only a few times a year. So even after you place your order it might take months before your source arrives. But that’s not really what you were asking – you were wondering where the isotopes come from, and that’s a lot more interesting!
The short version is that most synthetic nuclides are made in one of two ways – splitting really large atoms such as uranium or adding neutrons to a stable atom. Let’s start with the second one first.
Say I have a piece of cobalt lying around (I mean, who doesn’t, right?) and I put it into a nuclear reactor. Flying around inside the reactor are countless neutrons from all the uranium fission that’s taking place. Every single cobalt atom on Earth has a nucleus that holds 27 protons and 32 neutrons. So what happens if one of those neutrons flying around in the reactor core hits a cobalt atom? Well…there’s a fairly reasonable chance that it will “stick” – that it will be absorbed by the nucleus, which will now have 33 neutrons to complement its 27 protons. Or, put another way, my stable Co-59 atom will have turned into radioactive Co-60. This is what’s called a neutron activation product because adding a neutron caused it to become activated. This is where most of the world’s Co-60 comes from.
Another way to cause a stable atom to become radioactive is to put it into the beam of a particle accelerator – what happens here depends on the type of the accelerator. If it’s slinging high-energy electrons around then one of those electrons might knock a proton or neutron out of the nucleus, causing an imbalance in the forces within the nucleus that will lead it to become radioactive. It’s also possible that some of the neutrons knocked out by electrons (or by high-energy photons that are also produced by such high-energy electrons) might be captured by another atom causing the now-familiar neutron activation. Or the accelerator could be throwing positive ions around, slamming the atoms with alpha particles or with protons. Whatever the particle, though, the end result is more or less the same – some of the atoms in the line of fire will end up with a different number of protons and neutrons in the nucleus and that will cause the atom to become radioactive. These are all called activation products (a general term that includes neutron activation), and this is one of the main ways of producing isotopes.
I should mention, too, that we can add neutrons to atoms that are already unstable to produce heavier and (to me, anyhow) more interesting radioactive atoms. The plutonium in nuclear weapons comes from adding a neutron to a U-238 atom to form U-239, which undergoes two beta decays to form Pu-239. Americium-241 (like what’s in your smoke detectors) can be produced this way as well, as can the californium (Cf-252) used to make some neutron sources.
So – that’s how all of the activation products are made; by putting a target atom into a neutron (or other particle)-rich environment and bombarding it to produce the nuclide(s) you’re interested in. But there’s an even more fun way to do it, and this also involves nuclear reactors.
If you split an atom of U-235 with a neutron it will form two radioactive fission fragments – about 5-6% of the time one of these fragments will have an atomic mass of 137. Depending on the exact number of protons it might be an atom of barium, cesium, xenon, or any other element that can hold 137 protons and neutrons together for a short period of time, and then it decays away, usually by giving off a beta particle. When that happens it turns into a different element (for example, Cs-137 gives off a beta particle to turn into Ba-137). Crucially, this decay product is likely to have a longer half-life than the original nuclide, and whatever it decays into will likely have a half-life that’s longer still. This process continues until it reaches cesium – with a half-life of about 30 years, Cs-137 accumulates faster than it decays away. When enough Cs-137 (or whatever they’re interested in) has been produced it can be removed and made into radioactive sources or compounds.
One way to get the Cs-137 out is to simply take the reactor fuel out of the reactor, dissolve it in acid, and chemically extract the cesium from the fuel. But spent reactor fuel has a lot of radionuclides in it, making it very radiologically “hot,” so it needs to spend anywhere from several months to a few years in a pool of water to cool off to the point where it can be processed. Plus, fuel rods are long and unwieldy and they contain a lot of metals in addition to the uranium and fission products. For this reason, a lot of fission product nuclides are produced by inserting fissionable U-235 into the reactor core, not as part of the fuel, but as part of a small package whose purpose is to produce fission products, not power. As the package sits in the reactor core absorbing neutrons many of those uranium atoms are fissioning away, and 5-6% of them produce atoms that end up as Cs-137. It still has to be chemically processed to extract the cesium – but it’s a lot easier to work with the small package than with the entire fuel rod. Oh – and it’s still highly radioactive, enough so that it can only be safely worked on in a hot cell surrounded by thick leaded glass. But it’s still safer than working on a fuel rod.
In both cases, producing the nuclides (whether by fission or activation) and extracting them from the fissionable materials is the start of the process; once extracted they need to be chemically processed to get them into the appropriate chemical form – for some (cobalt or iridium for example) they’re usually used in metallic form, others (e.g. strontium) are most useful as ceramics, medical nuclides might be turned into simple compounds (the I-131 used to treat thyroid cancer is usually in the form of sodium iodide), and so forth. Finally, once the material is in the proper chemical form it’s packaged in the appropriate physical form – as a liquid radiopharmaceutical, a solid radioactive source inside a metal capsule, and so forth according to its intended use.
So that’s where all the non-NORM nuclides come from! Fissioned or bombarded, chemically processed, and then sealed into tidy little packages for our use.
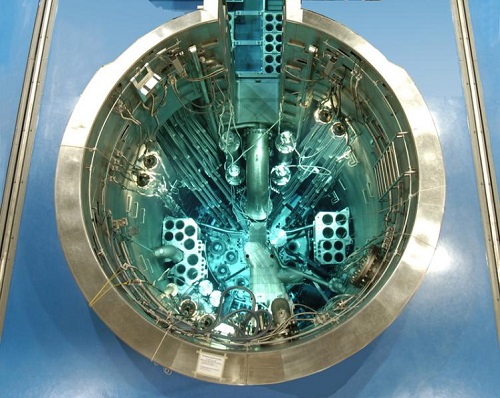
I guess one other tidbit is worth mentioning, too. Some isotopes are produced at only one or two reactors around the world and if the production reactor has to shut down, say, for refueling or maintenance, then global production of that nuclide can slow or even stop. Politics, too, can come into play if the sole source of production is in a country that becomes outcast or that decides to stop selling nuclides to a rival. Transportation issues can also make it hard to get a particular nuclide, as can any of a number of other supply chain issues. So producing the nuclides is often the easy part – getting them to the customer can often be far more of a challenge.